±è°ü¼®
|
2024-08-03 08:46:04, Á¶È¸¼ö : 6,584 |
- Download #1 : Baumann_1ts.jpg (625.2 KB), Download : 5
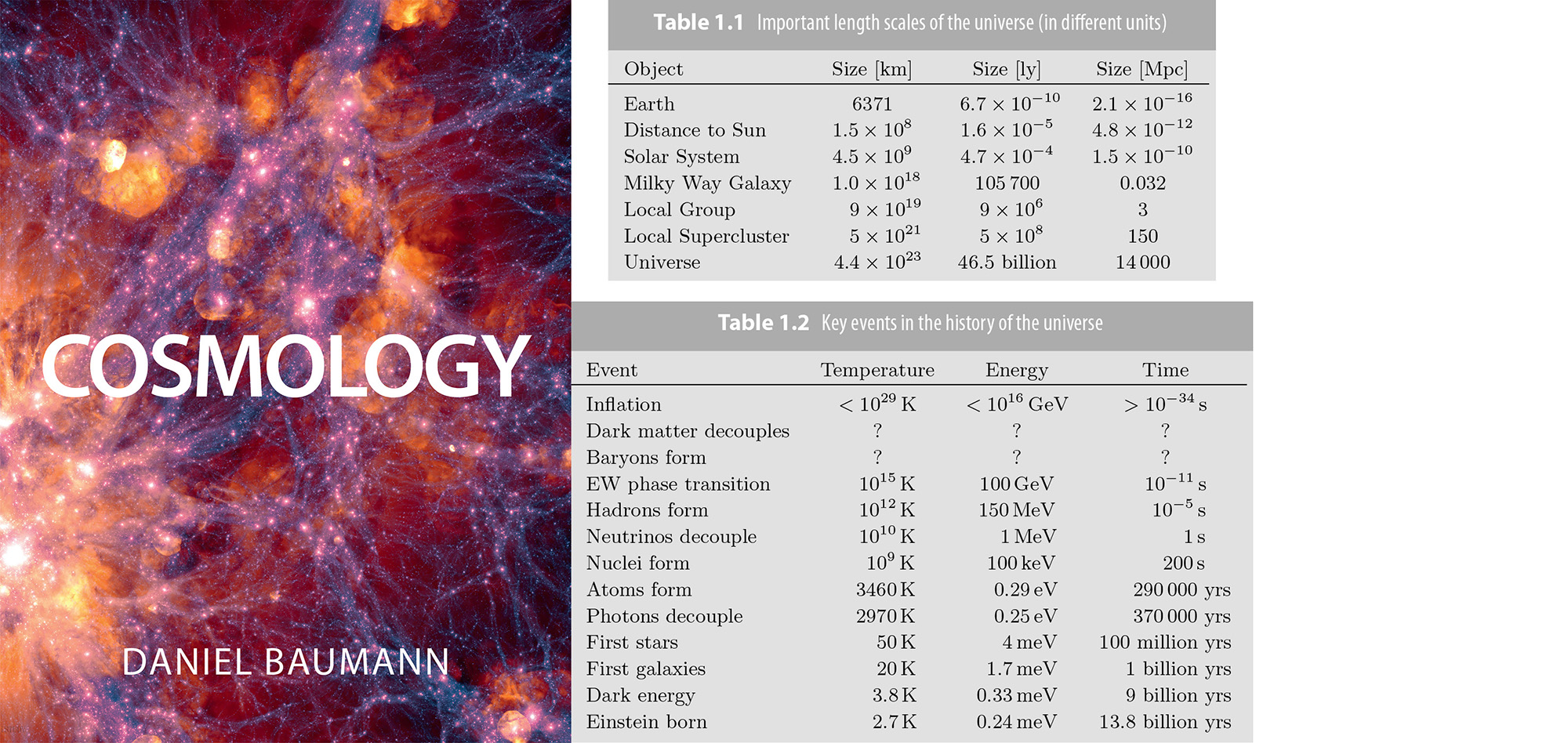

..... A century ago, we didn't know that there are other galaxies beyond our own .....
..... Even just twenty-five year ago, we didn't know what most of the universe is made of .....
..... Finally, we have found evidence that the primordial density perturbations originated from microscopic quantum fluctuations .....
1 Introduction
This book is about 13.8 billion years of cosmic evolution. We will trace the history of the universe from fraction of a second after the Big Bang until today. Before we begin our journey, however, it will be useful to have a bird's eye view of the subject. In this chapter, we will set the stage by giving a qualitative account of the structure and evolution of the universe, as we now understand it. In rest of the book, we will see where this knowledge comes from.
1.1 Scale of the Universe
The length scales in cosmology are so enormous that they are hard to grasp (see Table 1.1). Evolution simply hasn't equipped the human brain with the ability to have any intuition for the vastness of the universe.
Let us start with the Solar System. The size(radius) of Earth is 6371 km. Light makes the trip to the Moon in just 1.3 seconds and it does to the Sun over 8 minutes.
(1.1) 1 ly ≈ 9.5 ¡¿ 1015 m.
Astronomers also use parsecs (pc). [Wikipedia Parsec]
(1.2) 1 pc ≈ 3.26 ly.
The nearest star, Proxima Centauri, is 4.2 light-years (or 1.3 pc) away.
Our Galaxy, the Milky Way, contains about 100 billion stars arranged in a flattened disc. It is about 30 kpc or 100,000 ly across. The Sun is located at the edge of a spiral arm, 30,000 light-years from the center. It takes 250 million years for the Sun to complete one orbit around the center of the Galaxy.
There are about 100 billion galaxies in the observable universe, each with about 100 billion stars.Our nearest neighbor is the Andromeda Galaxy (M31) which is one of about fifty nearby galaxies that are gravitationally bound together. This arrangement of galaxies, called the Local Group, spans about 10 million light-years.
On even largest scales, galaxies arrange themselves into clusters and superclusters, with filamentary structures and giant voids in between them. The largest such structure, like our Local Supercluster (Laniakea), are about 500 million light-years across.
Because the universe has a finite age, there is a maximum distance that we can see. The radius of the observable universe is
(1.3) 46.5 billion ly ≈ 14 Gpc ≈ 4.4 ¡¿ 1026 m.
Notice that this larger than the naive distance that light traveled in the age of the universe which is 13.8 billion years. This discrepancy arises because the universe is expanding and the light is carried along the expansion.
1.2 The Invisible Universe
Most of the matter and energy in the universe is invisible. The stuff that we can see-ordinary atoms-accounts for less than 5% of the total. The rest is in the form of dark matter and dark energy.
The majority of mass in the universe is composed of dark matter. Since Fritz Zwicky in 1933 found "dunkle materie" seemed to be required to hold the Coma cluster together and Vera Rubin and collaborators in 1970s measured the large rotation speeds of hydrogen gas in the outer reaches of galaxies which could only be explained if these galaxies were embedded in halos of matter.
Today, some of the most striking evidence for dark matter comes from the gravitational lensing of the cosmic microwave background (CMB). As the CMB photons travel through the universe, they get deflected by the intervening large-scale structure, which distorts the hot and cold spot of the CMB. The effect depends on the total amount of matter in the universe and has been measured by the Planck satellite. In particular, the small density variations observed in the early universe only grow fast enough if assisted by the gravitational pull of dark matter. The true nature of putative dark matter remains a pressing open problem in astrophysics and cosmology.
In the 1980s, cosmology was in crisis, the age of a matter-only universe seemed to be shorter than the ages of the oldest stars within it. In addition, observations of the large-scale clustering of galaxies implied that the total matter density was only around 30% of the critical density required for a spatially flat universe, in conflict with the theoretical expectation from inflationary cosmology. To account this "missing energy," some theorists suggested the existence of a new form of dark energy, but direct evidence for it was lacking. Both problems were resolved with the discovery of the accelerating universe.By observing distant supernova explosions, cosmologists were able to measure both the distance and the recession speeds of far-away galaxies. The result showed that the rate of expansion was decelerating at early times, but started to accelerate a few billion years ago. The accelerated expansion increases the estimate for the age of the universe, reconciling it with an ages of the oldest stars. Moreover, shortly after the supernova observations, balloon-borne CMB experiments provided strong observational evidence for spatial flatness of the universe and hence solidified the case that dark energy was needed to explain 70% of the total energy in the universe today. But we don't know what it is. Such a "vacuum energy" is predicted by quantum field theory, but its estimated size is many orders of magnitude larger than the observed dark energy density. Explaining this discrepancy remains one of the biggest open problems in cosmology and fundamental physics.
1.3 The Hot Big Bang
The universe is expanding. It was therefore denser and hotter in the past. Particles were colliding frequently and the universe was in a state of thermal equilibrium with an associated temperature 𝑇. We will often use the particle physicists' convention of measuring energies in electron volt (eV):
(1.4) 1 eV ≈ 1.60 ¡¿ 10-19 J ≈ 1.16 ¡¿ 104 K. [Wikipedia Electronvolt: 1 eV/𝑘B ≈ (1.60 ¡¿ -19 J)/(1.38 ¡¿ -23 j/K) ≈ 1.16 ¡¿ 104 K]
A useful relation between the temperature of the early universe and its age is
(1.5) 𝑇/(1 MeV) ≈ (𝑡/1 s)-1/2. [RE (3.56) p.85: We can get this from the Friedmann equation. It is a useful rule of thumb that the temperature of the universe 1 second after the Big Bang was about 1 MeV (or 104 K), and evolved as 𝑡-1/2 before that.]
While there was very little time available in the early universe, the rates of reactions were extremely high, so that many interesting things happen in a short amount of time (see Table 1.2).
Above 100GeV (or 10-12 s), all particles of the Standard Model were in equilibrium and were therefore present in roughly equal abundances. This state can be viewed as the initial condition for the hot Big Bang. The density was 1036 kg/cm3.[citation needed] In 10-6 s, the universe expanded by a factor of 1026. *[correction: 104 ¡æ 1026] During this expansion, the temperature dropped and the universe went through different evolutionary stages.[Wikipedia Chronology of the universe<; Planck epoch: < 10-43 s; Grand Unification epoch: 10-43 s ~ 10-36 s; Inflationary epoch: < 10-32 s The universe is thought to have expanded by a factor of at least 1078 in volume - 1026 in length - equivalent to 10-9 m molecule expanding to one 10.6 light-years long]
At around 100 GeV (or 1015 K), the electroweak (EW) symmetry of the Standard Model was broken during the EW phase transition. Although the basics of EV symmetry breaking are well understood-and have been experimentally verified by the discovery of the Higgs boson-the detailed dynamics of the EW phase transition and its observational consequences are still a topic of active research.
Once the temperature drops below the mass of a particle species,[citation needed] particles and antiparticles start to annihilate, while the reverse process - the spontaneous creation of particle-antiparticle pairs - becomes inefficient. The first particles to disappear from the universe in this way were top quarks. 𝑊 and 𝑍 bosons followed. Then the Higgs, the bottom and charm quarks and tau lepton. Around 150 MeV, the QCD phase transition occurred and the remaining quarks condensed into hadrons (mostly protons, neutrons and pions). [Wikipedia Chronology of the universe; Quark epoch and Hadron epoch]
Particles fall out of thermal equilibrium when their interaction rate drops below the expansion rate of the universe. At that moment, the particles stop interacting with the rest of the thermal bath and a relic abundance will be created.[citation needed] It is likely that the dark matter was created in this way. About one second after the Big Bang the decoupling of neutrinos which produced the cosmic neutrino background (C𝜈B) happened. During the early radiation-dominated period, these cosmic neutrinos carried about 40% of the total energy density and had a significant effect on the expansion of the universe.[citation needed] Imprint of the C𝜈B have recently been detected in both CMB and the clustering of galaxies.[citation needed] These observations provide a window into the universe when it was just one second old.
After QCD phase transition, the universe was a plasma of mostly free electrons, protons and neutrons, as well as very energetic photons that prevented any heavier nuclei from forming. About one minute after the Big Bang, the temperature had dropped enough for the synthesis of helium-4 and lithium-7 to become efficient. The predicted amounts of these elements are consistent with the abundances found in early gas clouds, where very little post-processing of the primordial abundances has taken place. Big Bang nucleosynthesis (BBN) produced very few nuclei that are heavier than lithium, because there are no stable nuclei with 5 or 8 nucleons that would be required to sustain the nuclear chain reactions. Heavier elements were instead produced in the interior of stars, where the high densities and long timescales involved allow for three helium-4 nuclei to fuse. These heavier elements include carbon and oxygen which were spread throughout the universe after the stars exploded.
About 370,000 years after the Big Bang, the universe had cooled enough the first stable atoms to form in a process called recombination. At that moment, light stopped scattering off the free electrons in the plasma and started to propagate freely through the universe.these free-streaming photons are still seen today as an afterglow of the Big Bang. stretched by 13.8 billion years of cosmic expansion, the universe's first light is observed as a faint microwave radiation - the cosmic microwave background. the CMB contains tiny variations in its intensity (as a function of direction on the sky) that reflect perturbations in the density of matter in the early universe. More than any other cosmological probe, the study of the CMB anisotropies has transformed cosmology into a precision science.
What had been described so far are either facts (like BBN and recombination) or theoretical extrapolations that we can make extremely high confidence (like the EW and QCD transitions). However, there are two important events in the early universe that we know must occurred, but whose details we are much less certain of. the first is dark matter production. some process must have led to the abundance of dark matter observed in the universe today. For example, weakly interacting massive particles (WIMPs) could have decoupled from the primordial plasma at high energies or a massive boson (maybe an axion) could have stared to oscillate around minimum of its potential when the expansion rate dropped below the mass of the particle, producing a bosonic condensate. The nature of the dark matter and its production in the early universe remain important open problems
Another event that we believe must have occurred in the early universe, but whose details are unknown is baryogenesis. This refers to the mechanism by which an asymmetry was created between matter and antimatter. The required asymmetry is very small: for every 1010 particles of antimatter there must have been one extra matter particle. In this way, the annihilation between matter and antimatter in to photons produces the observed matter-to-photon ratio in the universe.There are many models of baryogenesis, but no way to decide which of these (if any) is the correct one.
1.4 Growth of Structure
The density fluctuations in the early universe eventually grew into all of the structure we around us. On large scales, the gravitational clustering of matter can be described analytically, while, on small scales, the process becomes highly nonlinear and only be captured by numerical simulations. The dark matter formed a web-like structure with high-density nodes connected by filaments. The baryonic gas collected in the regions of high dark matter where it collapsed into stars which then congregated into galaxies.
The first stars - called Population III stars - formed when the universe about 100 million years old. Computer simulations suggest that these stars were very massive, about a few hundred times more massive than the Sun. They were very luminous and burned up their fuel rapidly. Although the first stars were short-lived, they emitted ultraviolet light which heated and ionized the surrounding gas. The dynamics of this process of reionization are still not completely known and are actively investigated through numerical simulations. The first stars also may have provided the seeds for the growth of supermassive black holes which are found at the centers of most galaxies. And they created the first heavy elements in their interiors which were dispersed throughout the cosmos when they exploded. Enriched with these heavy elements, the baryonic gas cooled more efficiently, allowing smaller and more long-lived stars to be formed
The first galaxies started to appear about one billion years after the Big Bang. Over time, these galaxies formed clusters and superclusters, a process that is still ongoing today. In the future, however, the growth of structures will stop as dark energy starts to dominate the universe. In this book, we will be more interested in galaxies as tracers of the underlying distribution of dark matter, which in turn is determined by the seed fluctuations in the early universe. This distribution isn't random but has interesting spatial correlations which have been measured in large galaxy surveys.
1.5 Cosmic Palaeontology
We can only measure the spatial correlations between cosmological structures at late times. A central challenges of modern cosmology is to construct a consistent "history" of the universe that explains these correlations. This parallels the way palaeontologists infer the history of the Earth by studying the pattern of fossilized bones in the ground today.
A remarkable feature of the observed correlations in the CMB is that they span scales that are larger than the distance traveled by light between the beginning of the hot Big Bang and the time when the CMB was created. This is in conflict with causality, unless the correlations were generated before the hot big bang. Indeed, there is now growing evidence that the Big Bang was not the beginning of time, but that the primordial density fluctuations were produced during an earlier period of accelerated expansion called inflation. Small quantum fluctuations during inflation were amplitude were amplified by rapid expansion of the space and became the seeds for the large-scale structure of the universe.
If inflation really occurred, it was a rather dramatic event in the history of the universe. In just a 10-33 seconds, the universe expanded about 1024 times in size. [Wikipedia Cosmic inflation: Before 10-32 seconds after the Big Bang the universe expand at least 1026 times in every spatial dimension.] A region of space the size of a mosquito got stretched to that of a galaxy. the observable universe then originated from a microscopic, causally-connected region of space.The correlation observed in the afterglow of the Big Bang were inherited from the correlations of the quantum fluctuations during inflation. It must be emphasized that inflation is not yet a fact, but BBN is a fact for example. The theoretical framework of inflation is sufficiently well developed to justify including it in many introductory textbook on standard cosmology. Moreover, many new observations of the primordial correlations are carried out that will subject the inflationary paradigm to further test.
Problems
1.1 The range of length scales involved in cosmology is hard to grasp. The best we can do is to consider relative distance and compare them o something familiar. In this exercise we will make some attempt to obtaining a more intuitive understanding of vastness of the cosmos.
1. Consider shrinking the Earth to the size of a basketball (24cm). What would then be the size of the Moon and its orbit around the Earth?
[Solution] The Moon would be the size of a baseball (7.5cm) and its orbit would be that of about a basketball court (29 ¡¿ 15m). ▮
2. Imagine scaling the Earth down to the size of a peppercorn (3mm). What would be the size of the Sun and the Earth's orbit? How far away would be the most distant planet in the Solar system be?
[Solution] The Sun would be the size of a basketball and Earth's orbit about twice the basketball court. The most distant planet, Neptune, would be about one kilometer. ▮
3. The "Solar Neighborhood" is a collection of about fifty nearby stars spread across about 65 ly, that travel together with the Sun. Scaling this region down to a basketball court, what would be the size of the solar system?
[Solution] The solar system would be the size of a grain of medium sand (0.3mm). ▮
4. Shrinking our Galaxy to the size of the basketball court, what would now be the size of the Solar Neighborhood?
[Solution] The Solar Neighborhood would now be the size of a peppercorn. ▮
5. The "Local Group" comprises about fifty nearby galaxies, spread across about 10 million ly. If we squeeze this region into the size of the basketball court, what would be the size of our Milky Way galaxy.
[Solution] Our Milky Way galaxy would be size of a sponge cake (12cm). ▮
6. The largest structures in the universe, like our "Local Supercluster" are about 500 million ly across. scaling these superclusters down to the dimensions of the basketball court, what would be the size of our Local Group?
[Solution] The Local Group would become the size of a basketball (24cm). ▮
7. The radius of the observable universe is 46.5 billion ly. Compressing the observable universe to the size of basketball court, what would be the size of the largest superclusters?
[Solution] The largest superclusters would be the size of a cookie (5cm). ▮
1.2 A key parameter in cosmology is the Hubble constant
(1.2) 𝐻0 ≈ 70 km s-1 Mpc-1.
In the following, using the the measured value of Hubble constant, we will estimate a few fundamental scales of our universe.
1. What is the Hubbble time 𝑡𝐻0 ¡Õ 𝐻0-1 in years? This is the rough estimate of the age of the universe.
[Solution] The Hubble time is
(a) 𝑡𝐻0 ¡Õ 1/𝐻0 = Mpc/(70 km s-1) = (106 ¡¿ 3.26 ¡¿ 𝑐 ¡¿ yrs)/70 km s-1 = (3.26 ¡¿ 106 ¡¿ 3 ¡¿ 105)/70 yrs ≈ 14 billion yrs. ▮
2. What is the Hubble distance 𝑑𝐻0 ¡Õ 𝑐𝐻0-1 in meters?
[Solution] The Hubble distance is
(a) 𝑑𝐻0 ¡Õ 𝑐𝑡𝐻0 = 3 ¡¿ 108 m s-1 ¡¿ 14 ¡¿ 109 ¡¿ 3.1536 ¡¿ 107 s ~ 1026 m. ▮
3. The average density of the universe today is
(1.2.2) 𝜌0 = 3𝐻02/8¥ð𝐺
where 𝐺 = 6.67 ¡¿ 10-11 m3 kg-1 s-2 is Newton's constant. What is 𝜌0 in g cm-3? How does this compare to the density of water?
[Solution] The average density of the universe today is
(a) 𝜌0 = 3𝐻02/8¥ð𝐺 = 𝑐2 ¡¿ 3/𝑑𝐻0 ¡¿ 1/8¥ð𝐺 ≈ (3 ¡¿ 1010 cm s-1)2 ¡¿ [3/(1028 cm)2] ¡¿ 1/(6.67 ¡¿ 10-8 cm3 g-1 s-2) ~ 10-29 g cm3.
Since the density of the water, 𝜌𝐻2𝑂 = 1 g cm-3
(b) 𝜌0/𝜌𝐻2𝑂 ≈ 10-29. ▮
4. Let us assume that the universe is filled with only hydrogen atoms. What is then the total number of atoms in the universe? How does this compared to the number of hydrogen nuclei in our brain?
Hint: Assume that the brain is mostly water. Use 𝑚𝐻 ≈ 2 ¡¿ 10-24 g and 𝑚𝐻2𝑂 ≈ 3 ¡¿ 10-23 g.
[Solution] The number of atoms in the universe is
(a) 𝑁𝐻 = 𝜌0𝑉universe/𝑚𝐻 = (10-29 g cm-3)/(2 ¡¿ 10-24g) ¡¿ 4¥ð/3 (𝑑𝐻0)3 ≈ 2 ¡¿ 10-5 cm-3 ¡¿ (1028)3 cm3 ~ 1079
Since the volume of brain about 1000 cm3. the number of hydrogen nuclei in the brain is
(b) 𝑁𝐻 = 20 ¡¿ 𝑁𝐻2𝑂 = 20 ¡¿ 𝜌brain/𝑚𝐻2𝑂 𝑉brain = 20 ¡¿ 1 g cm-3 ¡¿ (103 cm3)/(3 ¡¿ 1023 g) ~ 1026
Comparing the number of the hydrogen nuclei in the brain to to those of the universe,
(c) 𝑁𝐻 universe/𝑁𝐻 brain ~ 1053. ▮
5. The maximal energy scale probed by the Large Hardron Collider (LHC) is 𝐸max ~10 TeV. What length scale 𝓁min does this the correspond to? How does the this compare to the size if the universe 𝑑𝐻0?
[Solution] The minimal length scale probed by 10 TeV LHC is
(a) 𝓁min = 𝘩𝑐/𝐸max = (1.2 ¡¿ 10-6 eV m)/(10 ¡¿ 1012 eV) ≈ 10-19 m
Comparing the minimal length scale probed by LHC to the size if the universe
(b) 𝑑𝐻0/𝓁min ~ 1026 m/10-19 m ~ 1045. ▮
* Textbook: Daniel Baumann, Cosmology. Cambridge University Press, 2022. (version 3, April 2023)
Major References (the abbreviations will be used)
• [Dodelson] S. Dodelson, Modern Cosmology. Academic Press, 2003.
• [Weinberg] S. Weinberg, Cosmology. Oxford University Press, 2008.
p.s. ±Ù·¡¿¡ ±¸ÇÑ BaumannÀÇ Cosmology´Â ¼¼°èÀû Àαâ Àü°øÀÚ Lecture Notes, CosmologyÀÇ ÃâÆÇº»ÀÔ´Ï´Ù.
¸çÄ¥µ¿¾È »ìÆìº¸°í ReferenceµéÀ» °ËÅäÇÑ °á°ú, 4³â ÀüÀÎ 2020³â¿¡ ÀϴܶôÇÑ ¿ìÁÖ·Ð ÇнÀÀ» Àç°³Çϱâ·Î Çß½À´Ï´Ù~
BaumannÀº 2008³â Princeton ´ë¿¡¼ Aspect of Inflation in String Theory (PhD ³í¹®)¸¦ ¾´ ÈÄ, 2011~2015³â Cambridge ´ë
Reader¸¦ ¿ªÀÓÇÑ ÈÄ, ÇöÀç´Â Amsterdam ´ë ±³¼ö·Î¼ Cosmology ¹× GRÀ» °ÀÇÇÏ´Â ¾ÆÁÖ(?) ÀþÀº À̷й°¸®ÇÐÀÚÀÌÀÚ ¿ìÁÖ·ÐÀÚÀÔ´Ï´Ù.
¼ö³â Àü, B. RydenÀÇ Introduction to Cosmology (Second edition. Cambridge University Press, 2017)À» °í·ÁÇÑ ÀûÀÌ ÀÖ¾úÁö¸¸ .....
±× ³»¿ëÀÌ ¿ìÁÖ·ÐÀ» ¼öÇÐÀ¸·Î ±â¼úÇϰí ÇаèÀÇ Æò°¡´Â ÁÁÀºµ¥, »ìÆìº¸´Ï±î, ¾Æ¹«·± Áõ¸íÀ̳ª Âü°í ¹®ÇåÀÌ ¾ø¾î¼ Àú¿¡°Ô´Â ¹«¿ëÁö¹°ÀÌ¿´³×¿ä..¤¾
¹Ý¸é¿¡, ÀÌ Ã¥Àº ÃֽŠ¿¬±¸±îÁö ¸Á¶óÇÏ°í ¼ö¸® Àü°³ °úÁ¤ ¹× Âü°í ¹®ÇåµéÀ» Ãæ½ÇÇÏ°Ô º¸¿©ÁֹǷΠself-study¿¡ ¾ÆÁÖ ÀûÇÕÇØ º¸ÀÔ´Ï´Ù!
'Mathematical Cosmology'ÀÇ ¿¬Àå¼±¿¡¼, ¿ä¾àÇÏ¸ç ¸®ºäÇÏ´Â ½ÄÀ¸·Î, ÀÌ Ã·´ÜÀÇ 'Precision Cosmology'¸¦ ޱ¸ÇÏ·Á°í ÇÕ´Ï´Ù.
Âü°í·Î, ¹ÌÀûºÐ ¹æÁ¤½ÄÀ̳ª °è»ê ¹®Á¦´Â WolframAlpha¸¦ »ç¿ëÇÕ´Ï´Ù. ÇлýÀ̶ó¸é Mathematica¸¦ »ç¿ëÇϰÚÁö¸¸ ...
[PDF·Î ¹èÆ÷µÈ Ä®¶ó figureµé°ú ¼±º°ÇÑ ¹®Á¦µéÀÇ derivation/solutionÀ» Æ÷ÇÔÇϸç, ¿Ï·á´Â ▮·Î Ç¥½ÃÇÔ.]
|
|
|